The role of gut microbiota metabolite trimethylamine N-oxide in functional impairment of bone marrow mesenchymal stem cells in osteoporosis disease
Introduction
Osteoporosis (OP) is a systemic disorder characterized by decreased bone density, destruction of bone microstructures, increased brittleness, and fracture risk (1). It is a significant public health challenge with potentially devastating results and a high cumulative rate of fractures (2). Recent studies have found that OP maythe kind of disease that is caused by an imbalance of stem cell regulation, especially in the pathogenesis of age-related OP (3). Bone marrow mesenchyme stem cells (BMSCs) are a type of stem cell widely existing in the bone marrow cavity, and have the potential to differentiate into various types of bone cells and secrete a variety of multifunctional cells that regulate bone metabolism (4). Studies have increasingly demonstrated that the function of BMSCs change changed in the pathological state of the body, which leads to the imbalance of bone metabolism and eventually contributes to OP (5).
Recently, the gut microbiota has attracted attention regarding its connection with metabolic diseases (6). These microbes have been considered as a key regulatory factor for metabolic disorders including obesity (7), diabetes (8), and OP (9). Growing numbers of studies have reported that gut microbiota regulate bone mass through immune system mediation (e.g., osteoclast genesis), intestinal calcium absorption, and the release of neurotransmitters (e.g., serotonin) (10,11). A better understanding of how microbes change structure and function will help us search for novel biomarkers and understand the relationship with bone mass disorder.
Trimethylamine-N-oxide (TMAO), a gut microbial-dependent metabolite of dietary choline, has been demonstrated to positively correlate with cardiovascular risks (12). A recent study found that TMAO is closely associated with age-related OP. Serum levels of TMAO were significantly higher in mice from the advanced age group compared to those from the normal group (13). Furthermore, recent clinical research demonstrated that TMAO was associated with bone mineral density (BMD) in patients with type 2 diabetes (14). However, there have been few studies regarding how TMAO affects bone metabolism, as well as the underlying molecular mechanisms.
In this study, we firstly analyzed TMAO concentration in the serum of OP patients and normal people, and found a relationship to be present between TMAO and the occurrence and development of OP. We continued by observing the effects of TMAO on BMSCs functions including cell proliferation, cell differentiation, cell metabolism, cell information transmission, et al. and the underlying mechanisms behind these effects. These results led to a deepening of understanding of how TMAO affects BMSCs, and provided evidence for the interaction between TMAO and bone mass disorder.
We present the following article in accordance with the MDAR reporting checklist (available at http://dx.doi.org/10.21037/atm-20-5307).
Methods
Clinical samples
We collected over 20 reports of menopausal patients who were examined physically or had BMD checked during their stay in the Department of Spinal Surgery, Affiliated Hospital of Guangdong Medical University from October 2018 to August 2019. After ruling out other relevant metabolic diseases, 10 OP patients aged <70 (inclusive) with T-scores <−2.5 SD (inclusive) at the femoral neck were included in the experimental group. The control group included 10 healthy people with T-scores >−1.0 SD (inclusive). Fresh venous blood was collected and measured by human trimethylamine oxide (TMAO) ELISA kit.
All procedures performed in this study involving human participants were in accordance with the Declaration of Helsinki (as revised in 2013). The study was approved by the Medical Institutional Review Board of the Affiliate Hospital of Guangdong Medical University (approval number YJYS2020063). Because of the retrospective nature of the research, the requirement for informed consent was waived.
Chemicals and antibodies
TMAO (Cat# 317594, Sigma-Aldrich, Burlington, MA, USA),fetal bovine serum (FBS) (Cat# 12483020, Thermo Fisher Scientific, Waltham, MA, USA), alpha-modified Eagle’s medium (α-MEM) (Cat# SH302265.01, Hyclone, Logan, Utah, USA), adipogenic differentiation medium (Cat# RASMX-90031, Cyagen, Guangzhou, China),osteogenic differentiation medium (Cat# RASMX-90021, Cyagen, Guangzhou, China),reactive oxygen species (ROS) assaykit (Cat# KGT010-1, KeyGen Biotech, Nanjing, China), human trimethylamine oxide (TMAO) ELISA Kit (Cat# JL47698, Jianglaibio, Beijing, China). Enzyme-linked immunosorbent assay (ELISA) kits for tumor necrosis factor-alpha (TNF-α), interleukin (IL)-1β, IL-6 were purchased from CUSABIO Biotechnology Company (Wuhan, China). Antibodies against nuclear factor kappa-light-chain-enhancer of activated B cells (NF-κB)-p65 (Cat# ab16502) were procured from Abcam (Cambridge, MA, USA). Antibodies against peroxisome proliferator-activated receptor y (PPARγ) (Cat# AF6284), and runt-related transcription factor 2 (RUNX2) (Cat# AF0313) were obtained from Affinity Biosciences (Jiangsu, China).
Primary culture and characterization of BMSCs
Rat BMSCs were isolated and expanded as previously described (15). In brief, a six-week-old Sprague Dawley (SD) rat was sacrificed by cervical dislocation, and was then immersed in 75% ethanol for 5–10 minutes. Using sterile surgical instruments, we carefully separated the intact rat tibia and femur. Bone marrow cells were flushed and centrifuged in a 1.073 g/mL Percoll density gradient. Enriched cells were collected from the interface and transferred into culture flasks with α-MEM supplemented with 10% FBS and 1% penicillin-streptomycin-neomycin (PSN) (all from Gibco, NY, USA).
After seeding for 72 h, nonadherent cells were discarded, and adherent cells were washed twice with phosphate-buffered saline (PBS). Fresh complete medium was added and replaced every 3 d. Cells from passages 3–5 were used for subsequent experiments.
Cell proliferation assay
To determine the dose-effect of TMAO on cell viability from cell line BV2, cells were treated with different doses (0, 50, 100, 200, 400, 600 µM) of TMAO. Cell viability was assessed using the CCK8 solution (Beyotime, Shanghai, China) at five different time periods. The absorbance at 450 nm was detected using a microplate reader (Biotek, Winooski, CA, USA), in order to count each value and compile a proliferation curve.
ROS assay
The accumulation of intracellular ROS was detected using a ROS Assay Kit according to the manufacturer’s instructions. In brief, BMSCs cells were treated with different concentrations of TMAO for 48 h. Cells were then collected, washed twice with a serum-free cell culture medium, and incubated with dichlorodihydrofluorescein diacetate (DCFH-DA) at 37 °C for 30 min in the dark. After being washed 3 times with the serum-free cell culture medium to fully remove any DCFH-DA that did not enter the cells, the ROS levels were detected by a FACSCantoTM II flow cytometry (BD Biosciences, San Jose, CA, USA).
Adipogenic and osteogenic differentiation
BMSCs were seeded in 12-well plates and treated with osteogenic induction medium (OIM) containing 10−7 M dexamethasone, 10 mM β-glycerophosphate and 50 µM ascorbate-2-phosphate (all from Sigma Chemical Co, St Louis, MO, USA) or adipogenic induction medium (AIM) containing 10−6 M dexamethasone, 0.2 mM Indomethacin, 0.1 mg/mL insulin, 1 mM 3-isobutyl-1-methylxanthin (IBMX) (Sigma). On day 14, the differentiation capacity was assessed by staining with Alizarin Red S or Oil Red O (Cyagen Biosciences, Santa Clara, CA, USA) following the manufacturer’s instruction. In briefly, for calcium deposit formation, cells were washed with PBS and fixed with 70% ethanol for 15 min. Then, cells werestained with 0.5% Alizarin Red S (pH 4.1) for 10 min at room temperature and washed three times with distilled water. Orange-red staining indicated the position and intensity of calcium deposits. Intracellular calcium deposition was observed by a light microscope. For fat droplets formation, cells were washed with PBS and fixed with 10% formaldehyde for 30 min. After two washes in PBS, cells were stained for 1 h in freshly diluted Oil Red O solution. Then, the stain was removed and the cells were washed twice with PBS. Images of cell stained with Oil Red O were obtained using an inverted microscope.
Detection of pro-inflammatory cytokine concentrations in cell supernatant
BMSCs were treated either with or without TMAO for 12, 24 and 48 h, respectively, and then the cell supernatant was collected. Pro-inflammatory cytokines (IL-1β, IL-6 and TNF-α) were determined using commercial ELISA kits in accordance with the manufacturer’s stipulations.
Western blot analysis
After 48 h of cell stimulation, different groups of proteins were extracted using radio immuno precipitation assay(RIPA) (with PMSF), and protein concentrations were determined using a bicinchoninic acid (BCA) protein assay kit (Beyotime, Shanghai, China). Total protein (10 µg) was separated on 10% or 12% sodium dodecyl sulfate polyacrylamide gel electrophoresis (SDS-PAGE) and transferred to polyvinylidene fluoride (PVDF) membranes (Millipore Corp., USA). The membranes were then blocked in tris buffered saline (TBS) containing 5% non-fat dry milk for 1 h, after which they were incubated with primary antibodies against PPARγ (1:500), C/EBP-α (1:1,000), RUNX2 (1:1,000), OPN (1:1,000), NF-κB (1:1,000), and GAPDH (1:2,000) overnight at 4 °C. The membranes were then washed with TBST and incubated for 1 h with secondary antibodies. The antibody-reactive bands were detected using the Pierce ECL Western Blotting Substrate (Thermo Fisher Scientific, Waltham, MA,USA). Quantification of band intensity was analyzed using Image J software (version 1.48; National Institutes of Health, USA).
Statistical analysis
The data were obtained from at least three independent experiments and presented as mean ± standard deviation. Single factor analysis of variance (ANOVA) and the t-test were adopted for comparison between groups. The data were analyzed by SPSS 20.0 (SPSS Company, Chicago, IL, USA). The level of difference was considered to be statistically significant when P<0.05.
Results
Serum TMAO level in OP patients is often higher than it is in normal people, and there is a negative correlation with BMD
Clinical samples were collected in accordance with the current standard protocols. Serum samples of 10 OP patients and 10 normal people were used to measure TMAO concentration and BMD by ELISA kit and dual-energy X-ray bone densitometer, respectively. Correlation analysis was performed between the TMAO concentration and BMD. The results indicated that the concentration of TMAO had a significant negative correlation with BMD (R2=−0.518, P=0.019) (Figure 1A). Furthermore, compared to the normal group, TMAO concentration was significantly higher in the OP patients (P=0.007<0.01) (Figure 1B).
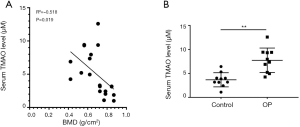
Effect of TMAO on BMSCs cell proliferation
The results from clinical samples have suggested that TMAO plays an important role in the development of OP, but its biological role requires further research. BMSCs were selected to be subjected to in vitro experiments. By examining how the TMAO levels changed in OP patients’ blood samples, we selected the appropriate TMAO concentration for subsequent exploration. In the cell proliferation experiment, the results showed that exposure to TMAO at the concentrations of 50 and 100 µM did not significantly influence cell viability, while the concentration of TMAO in the range of 200–600 µM significantly inhibited cell growth in a time- and dose-dependent manner (Figure 2).
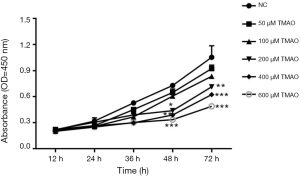
Effects of TMAO on ROS release and pro-inflammatory cytokine levels in BMSCs
TMAO treatment led to an increase in ROS formation in BMSCs, especially so when the level of TMAO was in the range of 200–600 µM (Figure 3A,B). Based on these results, the TMAO concentration of 200 µM was selected in the subsequent experiments. To elucidate the effects of TMAO on pro-inflammatory cytokine levels in BMSCs, cell supernatants were detected using commercial ELISA kits. The results demonstrated that the levels of IL-1β (P<0.001), IL-6 (P<0.001) and TNF-α (P<0.001) were significantly increased by TMAO treatment, when compared to the control group (Figure 3C,D,E).
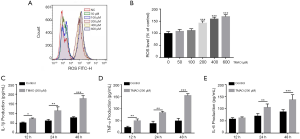
TMAO promotes the adipogenic differentiation and inhibits the osteogenic differentiation of BMSCs
To elucidate the effects of TMAO on adipogenic differentiation and osteogenic differentiation of BMSCs, we treated BMSCs with or without TMAO in adipogenic induction medium (AIM), or osteogenic induction medium (OIM). Oil red O staining was performed to evaluate the formation of fat droplets. Alizarin red staining was performed to evaluate calcium deposit formation. The results showed that cells treated with TMAO developed more fat droplets than the control cells did, and concurrently decreased mineralized nodules (Figure 4A,B). We further measured the corresponding osteogenic (Runx2 and OPN) or adipogenic (PPARγ and C/EBP-α) hallmark expression using western blotting. The results showed that TMAO increased the expression of PPARγ and C/EBP-α protein, and decreased the expression of Runx2 and OPN protein (Figure 4C,D). These results implied that TMAO could promote BMSCs adipogenic differentiation while it inhibited osteogenic differentiation.
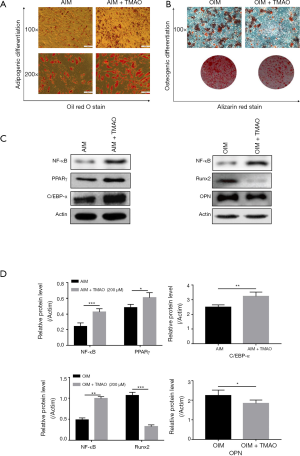
TMAO regulates BMSC functions via NF-κB signaling pathway
The NF-κB signaling pathway has been demonstrated to play a pivotal role in the regulation of bone metabolism (16). We assayed the protein level of NF-κB in order to identify whether the NF-κB signaling pathway participated in the TMAO-treated BMSC differentiation function. No matter whether under AIM or OIM, NF-κB protein expression levels in BMSCs were both increased in the presence of TMAO (Figure 4C,D). To confirm whether NF-κB activity was involved in TMAO-regulated BMSCs function, we applied BAY 11-7082, an inhibitor of NF-κB, to block the activity of NF-κB. As shown in Figure 5A and B, compared with the TMAO-treated group, BAY 11-7082 treatment significantly reduced the level of ROS. Similarly, ELISA results revealed that pretreatment with BAY 11-7082 markedly attenuated TMAO-induced pro-inflammatory cytokine levels (IL-1β, IL-6, and TNF-α) (Figure 5C,D,E). Moreover, Runx2 protein expression and mineralized nodules were significantly increased in the BAY 11-7082 treatment group, after the TMAO-treated BMSCs were in OIM (Figure 5F,G). In summary, these results suggested that NF-κB activity played a crucial role in TMAO-treated cell functions of BMSCs (Figure 5H).
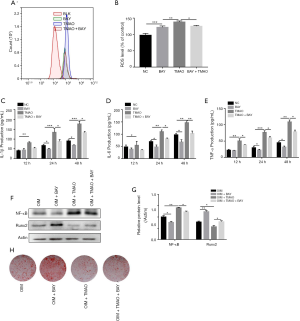
Discussion
OP is characterized by enhanced skeletal fragility due to the reduction of bone quantity and/or quality. Bone strength cannot be directly measured in vivo, but BMD is highly correlated with bone strength. Thus, BMD value is often used as a key clinical indicator to predict fracture risk (17). In this study, TMAO level showed a significant negative correlation with BMD value. Serum TMAO level in OP patients was higher than in the control group. These data indicate that TMAO plays an important role in the occurrence and development of OP.
Recent studies have shown that gut microbes can be key regulatory factors in bone physiology (18). The gut microbiota can activate inflammatory responses in various tissues including bone marrow by way of bacterial modifications, or through the actions of their metabolites (19). TMAO, a gut microbial-dependent metabolite of dietary choline, has been demonstrated to correlate with OP. In this study, cell proliferation was significantly decreased while the pro-inflammatory cytokine levels (IL-1β, TNF-α and IL-6) were significantly increased in BMSCs after treatment with TMAO. Furthermore, the intracellular ROS production increased in TMAO-treated BMSCs in a dose-dependent manner. These data indicated that the effects of TMAO on BMSCs function may be via the activation of inflammatory responses.
Currently, OP is widely considered to be a disease caused by the abnormal metabolism of BMSCs (20,21). Several lines of evidence have demonstrated that osteoporotic BMSCs have defects in intrinsic signals that cause functional alterations, leading to poor osteogenic differentiation capacity, and favoring increased adipogenesis (22). In this study, we found that TMAO could induce and promote the adipogenic differentiation of BMSCs, but inhibit the osteogenic differentiation of BMSCs. At the same time, the expression of adipogenic differentiation marker proteins PPARγ and C/EBP-α were up-regulated, and the expression of osteogenic differentiation protein Runx2 and OPN was down-regulated. These results confirmed that TMAO was involved in the development of OP by regulating the differentiation of BMSCs.
NF-κB is a master regulator of inflammation and the bone-remodeling process. Increased NF-κB activity is accompanied by enhanced bone-resorption and reduced bone-formation abilities (23). This study showed that NF-κB p65 expression was increased following TMAO treatment. To determine whether modulation of NF-κB was related to the TMAO-mediated BMSCs function, cells were pretreated with a specific inhibitor of NF-κB, BAY 11-7082,for 2 h, and then treated with TMAO. The results demonstrated that compared with TMAO treatment, NF-κB inhibitor BAY 11-7082 caused a significant decrease in pro-inflammatory cytokine (IL-1β, TNF-α and IL-6) production and ROS release in BMSCs. Furthermore, the expression of Runx2 (P<0.05) and calcium nodes were significantly reversed by BAY 11-7082 treatment.
However, there were several limitations in this study. Firstly, the sample size is relatively small; a larger sample size is needed for expansion of this research. Secondly, although this study demonstrated that TMAO modulates the function of BMSCs (including cell proliferation, cell differentiation, and inflammatory response) via the NF-κB signaling pathway, other signaling pathways (such as the autophagy signaling pathway) may also be involved, which requires further verification. Finally, how TMAO inhibits BMSCs osteogenic differentiation needs to be further verified in vivo study.
Conclusions
Our results have demonstrated that TMAO level is significantly negatively correlated with BMD value. TMAO decreases BMSCs proliferation, increases pro-inflammatory cytokine production and ROS release, inhibits osteogenic differentiation and promotes adipogenic differentiation by up-regulating the NF-κB signaling pathway, which may contribute to a bone metabolic imbalance, leading to aggravation of bone loss and further causation of OP.
Acknowledgments
Funding: This work was supported by the National Natural Science Foundation of China (81570260, 81600698); the Natural Science Foundation of Guangdong Province, China (2020A1515011565); the Medical Scientific Research Foundation of Guangdong Province, China (A2019463).
Footnote
Reporting Checklist: The authors have completed the MDAR reporting checklist. Available at http://dx.doi.org/10.21037/atm-20-5307
Data Sharing Statement: Available at http://dx.doi.org/10.21037/atm-20-5307
Conflicts of Interest: All authors have completed the ICMJE uniform disclosure form (available at http://dx.doi.org/10.21037/atm-20-5307). The authors have no conflicts of interest to declare.
Ethical Statement: The authors are accountable for all aspects of the work in ensuring that questions related to the accuracy or integrity of any part of the work are appropriately investigated and resolved. All procedures performed in this study involving human participants were in accordance with the Declaration of Helsinki (as revised in 2013). The study was approved by the Medical Institutional Review Board of the Affiliate Hospital of Guangdong Medical University (approval number YJYS2020063). Because of the retrospective nature of the research, the requirement for informed consent was waived.
Open Access Statement: This is an Open Access article distributed in accordance with the Creative Commons Attribution-NonCommercial-NoDerivs 4.0 International License (CC BY-NC-ND 4.0), which permits the non-commercial replication and distribution of the article with the strict proviso that no changes or edits are made and the original work is properly cited (including links to both the formal publication through the relevant DOI and the license). See: https://creativecommons.org/licenses/by-nc-nd/4.0/.
References
- Coughlan T, Dockery F. Osteoporosis and fracture risk in older people. Clin Med (Lond) 2014;14:187. [Crossref] [PubMed]
- Watts NB, Manson JE. Osteoporosis and fracture risk evaluation and management: shared decision making in clinical practice. JAMA 2017;317:253-4. [Crossref] [PubMed]
- Zhu Q, Shan C, Li L, et al. Differential expression of genes associated with hypoxia pathway on bone marrow stem cells in osteoporosis patients with different bone mass index. Ann Transl Med 2019;7:309. [Crossref] [PubMed]
- Wang C, Meng H, Wang X, et al. Differentiation of bone marrow mesenchymal stem cells in osteoblasts and adipocytes and its role in treatment of osteoporosis. Med Sci Monit 2016;22:226. [Crossref] [PubMed]
- Zhu Q, Shan C, Li L, et al. Differential expression of genes associated with hypoxia pathway on bone marrow stem cells in osteoporosis patients with different bone mass index. Ann Transl Med 2019;7:309. [Crossref] [PubMed]
- Arora T, Bäckhed F. The gut microbiota and metabolic disease: current understanding and future perspectives. J Intern Med 2016;280:339-49. [Crossref] [PubMed]
- Zhi C, Huang J, Wang J, et al. Connection between gut microbiome and the development of obesity. Eur J Clin Microbiol Infect Dis 2019;38:1987-98. [Crossref] [PubMed]
- Baothman OA, Zamzami MA, Taher I, et al. The role of gut microbiota in the development of obesity and diabetes. Lipids Health Dis 2016;15:108. [Crossref] [PubMed]
- Schepper JD, Collins F, Rios-Arce ND, et al. Involvement of the gut microbiota and barrier function in glucocorticoid induced osteoporosis. J Bone Miner Res 2020;35:801-20. [Crossref] [PubMed]
- McCabe L, Britton RA, Parameswaran N. Prebiotic and probiotic regulation of bone health: role of the intestine and its microbiome. Curr Osteoporos Rep 2015;13:363-71. [Crossref] [PubMed]
- Zhang J, Lu Y, Wang Y, et al. The impact of the intestinal microbiome on bone health. Intractable Rare Dis Res 2018;7:148-55. [Crossref] [PubMed]
- Randrianarisoa E, Lehn-Stefan A, Wang X, et al. Relationship of serum trimethylamine N-oxide (TMAO) levels with early atherosclerosis in humans. Sci Rep 2016;6:26745. [Crossref] [PubMed]
- Li L, Chen B, Zhu R, et al. Fructus LigustriLucidi preserves bone quality through the regulation of gut microbiota diversity, oxidative stress, TMAO and Sirt6 levels in aging mice. Aging (Albany NY) 2019;11:9348-68. [Crossref] [PubMed]
- Zhou T, Heianza Y, Chen Y, et al. Circulating Gut Microbiota Metabolite Trimethylamine N-Oxide (TMAO) and Changes in Bone Density in Response to Weight Loss Diets: The POUNDS Lost Trial. Diabetes Care 2019;42:1365-71. [Crossref] [PubMed]
- Wei B, Huang C, Zhao M, et al. Effect of mesenchymal stem cells and platelet-rich plasma on the bone healing of ovariectomized rats. Stem Cells Int 2016;2016:9458396.
- Novack DV. Role of NF-κB in the skeleton. Cell Res 2011;21:169-82. [Crossref] [PubMed]
- Lu Y, Genant HK, Shepherd J, et al. Classification of osteoporosis based on bone mineral densities. J Bone Miner Res 2001;16:901-10. [Crossref] [PubMed]
- Ding K, Hua F, Ding W. Gut Microbiome and Osteoporosis. Aging Dis 2020;11:438-47. [Crossref] [PubMed]
- Cammarota G, Ianiro G, Gasbarrini A. Fecal microbiota transplantation for the treatment of Clostridium difficile infection: a systematic review. J Clin Gastroenterol 2014;48:693-702. [Crossref] [PubMed]
- Jing H, Su X, Gao B, et al. Epigenetic inhibition of Wnt pathway suppresses osteogenic differentiation of BMSCs during osteoporosis. Cell Death Dis 2018;9:176. [Crossref] [PubMed]
- Liu Y, Wang X, Chang H, et al. Mongolian Medicine echinops prevented postmenopausal osteoporosis and induced ER/AKT/ERK pathway in BMSCs. Biosci Trends 2018;12:275-81. [Crossref] [PubMed]
- Phetfong J, Sanvoranart T, Nartprayut K, et al. Osteoporosis: the current status of mesenchymal stem cell-based therapy. Cell Mol Biol Lett 2016;21:12. [Crossref] [PubMed]
- Lin TH, Gibon E, Loi F, et al. Decreased osteogenesis in mesenchymal stem cells derived from the aged mouse is associated with enhanced NF-κB activity. J Orthop Res 2017;35:281-8. [Crossref] [PubMed]
(English Language Editor: J. Jones)