Esophageal electric fields are predictive of atrial cardioversion success—a finite element analysis
Introduction
Atrial fibrillation (AF) is the most common cardiac arrhythmia in the United States (1). It is characterized by disorganized cardiac electrical activity and an inability of the atria to effectively expel blood to the ventricles. One potential treatment of AF is external cardioversion, which includes the application of a strong electric shock through electrodes on the outside of the body. Studies have previously investigated the effect of electrode placement on cardioversion success, most attempting to find a universal, optimal configuration (2-7). The lack of agreement between these studies on a universal configuration suggests that patient-specific electrode placements may be the best way to optimize external cardioversion.
Two recent reports have described the results of preliminary studies investigating the role that esophageal electric fields (EEFs) could play in predicting patient-specific electrode placements (8,9). The first study reported a strong negative correlation between EEFs and atrial defibrillation thresholds (ADFTs) during preliminary computer simulations of 27 external electrode configurations (9). ADFTs are the minimum amount of energy required to successfully revert the heart to normal sinus rhythm. The second study reported negative correlations between EEFs during lower strength shocks (1 Joule) and ADFTs recorded in six pigs (8). These studies both suggest that EEFs measured in the portion of the esophagus adjacent to the atria could be used to distinguish between various electrode placements and predict a patient-specific optimal configuration.
While the results of these preliminary studies suggest using EEFs to predict patient-specific electrode placements is plausible, both are based upon small numbers of measurements. The objective of the current study was to determine if the previously reported relationship between EEFs and ADFTs holds during a series of computer simulations consisting of over 600 external electrode placements.
Methods
Finite-element computer model
A three-dimensional, anatomically realistic finite-element computer model of a male human torso that has previously been shown to accurately predict clinically a relevant difference in ADFTs was used (Figure 1) (10). The geometry for the model was obtained from 90 transverse magnetic resonance images (MRIs) taken of the torso of a 190 cm tall, 80 kg healthy male (10-12). Tracings were made of the following 14 anatomical regions: body surface, skeletal muscle, fat layer, left lung, right lung, epicardium, left atrium, right atrium, left ventricle, right ventricle, superior vena cava, inferior vena cava, pulmonary trunk, and aorta. The coordinates from the tracings were triangulated and tessellated into 377,127 tetrahedral elements that were classified as one of the 14 anatomical regions.
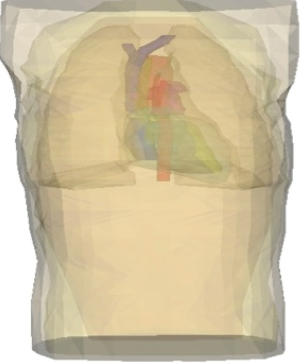
Simulations
Torso geometry, cardioversion electrode placements, and tissue conductivities (Table 1) served as inputs for the model. The simulated cardioversion electrodes were a clinically realistic size of 100 cm2. A total of 625 anterior-posterior (AP) electrode configurations were created by pairing a single electrode on the anterior chest with one on the posterior chest. Figure 2 shows the total area of the torso body surface covered by the cardioversion electrode pairs.
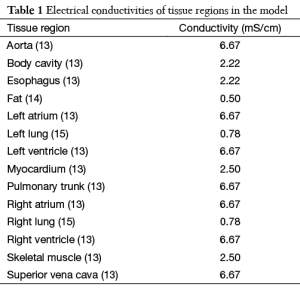
Full table
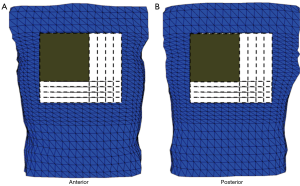
For each electrode placement, a 100 V potential difference was simulated across the cardioversion electrodes. An average electric field value was calculated for the tetrahedral elements of the esophagus directly adjacent to the atria. An ADFT was then calculated for the electrode placement by scaling the applied potential difference to produce a minimum potential gradient (5 V/cm) throughout a critical mass (95%) of the atrial myocardium, as in (10). The EEF was squared because energy is related to the square of voltage. These steps were repeated for each of the 625 electrode placements. Both the EEF2 and ADFT were then normalized. Linear regression was performed to determine the correlation between the normalized EEF2s and ADFTs.
Results
Figure 3 shows there was no relationship between normalized EEF2s and normalized ADFTs when considering all 625 electrode placements. It also shows that of these 625 electrode configurations, there was not a single optimal electrode placement. Instead there were multiple configurations that resulted in equally low ADFTs.
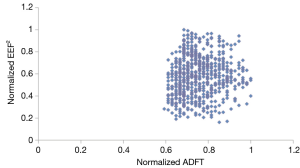
Since there was no correlation between normalized EEF2s and ADFTs for all placements, the authors next examined the correlation when small shifts, less than 2 cm, from clinically relevant electrode placements were performed. Electrode pairs were created by holding the central anterior electrode stationary and shifting the posterior in the same lateral row for a total of five electrode pairs (PL), shifting the posterior electrode in the same axial column (PA), or shifting the posterior electrode in a square pattern (PSQ). Similarly, electrode pairs were created by holding the central posterior electrode stationary, while shifting the anterior electrode in the same lateral row as the posterior (AL), shifting the anterior electrode in the same axial column as the posterior (AA), or shifting the anterior electrode in a square pattern (ASQ).
Linear regression was used to determine the coefficient of determination (R2) and correlation coefficient (R) between normalized EEF2s and ADFTs for each electrode-shifting pattern (Table 2). The percent difference between the maximum and minimum ADFT (ADFTmax-min) for each shifting pattern was also calculated. Figure 4A-D shows the relationships between normalized EEF2s and ADFTs for PL, PSQ, AL, and ASQ.

Full table
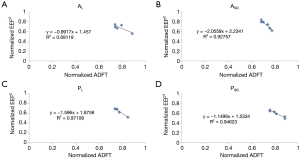
Discussion
The relationship between normalized EEF2s and ADFTs during external atrial cardioversion was investigated using an anatomically realistic, three-dimensional finite element computer model of the human torso. There was no correlation between normalized EEF2s and ADFTs for the 625 electrode configurations overall. These results show there may not be a single, optimal electrode placement for each patient but instead several electrode placements that result in equally low ADFTs. There was over 40% difference between the smallest and largest ADFTs or the most and least optimal electrode configurations. This means there is potential to reduce required energy levels up to 80 J when considering the suggested initial energy of 200 J (16).
The authors next investigated if small shifts (<2 cm) from clinically relevant electrode placements could be used to reduce ADFTs. There was a strong negative relationship between normalized EEF2s and ADFTs when the anterior electrode was held constant and the posterior electrode was shifted laterally in the same plane (R2 =0.971) or in a square pattern (R2 =0.946). There was a similarly strong negative relationship when the posterior electrode was held constant and the anterior electrode was shifted in a square pattern (R2 =0.927) and a less significant relationship (R2 =0.681) when the anterior electrode was shifted laterally in the same plane. There was no relationship when either electrode was shifted axially.
The described relationships may have potential application in the clinical setting. In practice, a clinician could position the external electrodes and apply a low-strength shock while measuring the EEF in the portion of the esophagus adjacent to the atria. The clinician could then reposition the electrodes, reapply the low-strength shock, and again measure the EEF. This could be repeated for several electrode configurations with the full strength cardioversion shock being delivered through the configuration that resulted in the largest EEF. The idea being the electrode placement resulting in the largest EEF adjacent to the atria during the low strength would result in the largest electric field in the atria during the cardioversion shock increasing the chance of success during the first shock and reducing overall delivered energy when multiple shocks are required.
The findings that there is not a single optimal electrode configuration and that small lateral or square electrode shifts had the highest correlation with ADFTs could simplify implementation of the described application. It would be unrealistic for a physician to iterate through all possible 625 electrode configurations to find a single optimal placement. Instead it is far more reasonable to start with a clinically relevant electrode placement and make small shifts. The results of these simulations show small shifts in a square pattern around the central posterior or anterior electrodes can result in up to a 13% reduction in ADFTs and lateral shifts can result in reductions of up to 14%.
Conclusions
In conclusion, a total of 625 electrodes configurations were simulated using a finite-element computer model of the human torso. There was no single optimal electrode configuration; instead multiple electrode placements resulted in similarly low ADFTs. Overall, there was no relationship between EEF2s and ADFTs, but there was a strong negative relationship when one electrode was shifted while holding the other electrode constant. These results suggest it is plausible to use EEFs to optimize external atrial cardioversion electrode placements using a small shift protocol.
Acknowledgements
None.
Footnote
Conflicts of Interest: The authors have no conflicts of interest to declare.
References
- Fuster V, Rydén LE, Cannom DS, et al. ACC/AHA/ESC 2006 Guidelines for the Management of Patients With Atrial Fibrillation: A Report of the American College of Cardiology/American Heart Association Task Force on Practice Guidelines and the European Society of Cardiology Committee for Practice Guidelines (Writing Committee to Revise the 2001 Guidelines for the Management of Patients With Atrial Fibrillation): Developed in Collaboration With the European Heart Rhythm Association and the Heart Rhythm Society. Circulation 2006;114:e257-354. [PubMed]
- Botto GL, Politi A, Bonini W, et al. External cardioversion of atrial fibrillation: role of paddle position on technical efficacy and energy requirements. Heart 1999;82:726-30. [PubMed]
- Kirchhof P, Eckardt L, Loh P, et al. Anterior-posterior versus anterior-lateral electrode positions for external cardioversion of atrial fibrillation: a randomised trial. Lancet 2002;360:1275-9. [PubMed]
- Mathew TP, Moore A, McIntyre M, et al. Randomised comparison of electrode positions for cardioversion of atrial fibrillation. Heart 1999;81:576-9. [PubMed]
- Mehdirad AA, Clem KL, Love CJ, et al. Improved clinical efficacy of external cardioversion by fluoroscopic electrode positioning and comparison to internal cardioversion in patients with atrial fibrillation. Pacing Clin Electrophysiol 1999;22:233-7. [PubMed]
- Vogiatzis IA, Sachpekidis V, Vogiatzis IM, et al. External cardioversion of atrial fibrillation: the role of electrode position on cardioversion success. Int J Cardiol 2009;137:e8-10. [PubMed]
- Zhang B, Li X, Shen D, et al. Anterior-posterior versus anterior-lateral electrode position for external electrical cardioversion of atrial fibrillation: a meta-analysis of randomized controlled trials. Arch Cardiovasc Dis 2014;107:280-90. [PubMed]
- Fitch DA, Soberman J, De Jongh Curry AL. Esophageal electric fields are predictive of atrial defibrillation thresholds. Pacing Clin Electrophysiol 2012;35:335-40. [PubMed]
- Fitch DA, Soberman JE, de Jongh Curry A. Esophageal electric fields are correlated to atrial defibrillation thresholds: towards patient-specific optimization of external atrial defibrillation. Conf Proc IEEE Eng Med Biol Soc 2006;1:4378-81. [PubMed]
- Hunt LC, de Jongh Curry AL. Electrode placement significantly affects transthoracic atrial defibrillation thresholds. Cardiovascular Engineering 2005;10:62-68.
- de Jongh AL, Entcheva EG, Replogle JA, et al. Defibrillation efficacy of different electrode placements in a human thorax model. Pacing Clin Electrophysiol 1999;22:152-7. [PubMed]
- Hunt LC, de Jongh Curry AL. Finite element computer modeling of transthoracic atrial defibrillation. Conf Proc IEEE Eng Med Biol Soc 2004;6:3964-7. [PubMed]
- RUSH S. ABILDSKOV JA, McFEER. Resistivity of body tissues at low frequencies. Circ Res 1963;12:40-50. [PubMed]
- Kaufman W, Johnston FD. The electrical conductivity of the tissues near the heart and its bearing on the distribution of the cardiac action currents. American Heart Journal 1943;26:42-54.
- Geddes LA, Baker LE. The specific resistance of biological material--a compendium of data for the biomedical engineer and physiologist. Med Biol Eng 1967;5:271-93. [PubMed]
- Wozakowska-Kaplon B, Janion M, Sielski J, et al. Efficacy of biphasic shock for transthoracic cardioversion of persistent atrial fibrillation: can we predict energy requirements? Pacing Clin Electrophysiol 2004;27:764-8. [PubMed]